As part of our Ask the Brain series, Halie Olson, a graduate student in the labs of John Gabrieli and Rebecca Saxe, pens her answer to the question,”Can I rewire my brain?”
_____
Yes, kind of, sometimes – it all depends on what you mean by “rewiring” the brain.
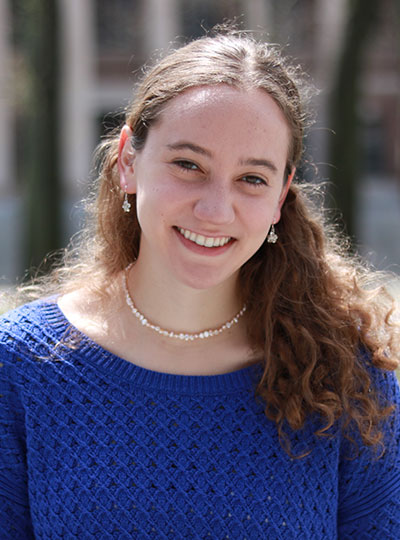
If you’re asking whether you can remove all memories of your ex from your head, then no. (That’s probably for the best – just watch Eternal Sunshine of the Spotless Mind.) However, if you’re asking whether you can teach a dog new tricks – that have a physical implementation in the brain – then yes.
To embrace the analogy that “rewiring” alludes to, let’s imagine you live in an old house with outlets in less-than-optimal locations. You really want your brand-new TV to be plugged in on the far side of the living room, but there is no outlet to be found. So you call up your electrician, she pops over, and moves some wires around in the living room wall to give you a new outlet. No sweat!
Local changes in neural connectivity happen throughout the lifespan. With over 100 billion neurons and 100 trillion connections – or synapses – between these neurons in the adult human brain, it is unsurprising that some pathways end up being more important than others. When we learn something new, the connections between relevant neurons communicating with each other are strengthened. To paraphrase Donald Hebb, one of the most influential psychologists of the twentieth century, “neurons that fire together, wire together” – by forming new synapses or more efficiently connecting the ones that are already there. This ability to rewire neural connections at a local level is a key feature of the brain, enabling us to tailor our neural infrastructure to our needs.
Plasticity in our brain allows us to learn, adjust, and thrive in our environments.
We can also see this plasticity in the brain at a larger scale. My favorite example of “rewiring” in the brain is when children learn to read. Our brains did not evolve to enable us to read – there is no built-in “reading region” that magically comes online when a child enters school. However, if you stick a proficient reader in an MRI scanner, you will see a region in the left lateral occipitotemporal sulcus (that is, the back bottom left of your cortex) that is particularly active when you read written text. Before children learn to read, this region – known as the visual word form area – is not exceptionally interested in words, but as children get acquainted with written language and start connecting letters with sounds, it becomes selective for familiar written language – no matter the font, CaPItaLIZation, or size.
Now, let’s say that you wake up in the middle of the night with a desire to move your oven and stovetop from the kitchen into your swanky new living room with the TV. You call up your electrician – she tells you this is impossible, and to stop calling her in the middle of the night.
Similarly, your brain comes with a particular infrastructure – a floorplan, let’s call it – that cannot be easily adjusted when you are an adult. Large lesions tend to have large consequences. For instance, an adult who suffers a serious stroke in their left hemisphere will likely struggle with language, a condition called aphasia. Young children’s brains, on the other hand, can sometimes rewire in profound ways. An entire half of the brain can be damaged early on with minimal functional consequences. So if you’re going for a remodel? Better do it really early.
Plasticity in our brain allows us to learn, adjust, and thrive in our environments. It also gives neuroscientists like me something to study – since clearly I would fail as an electrician.
Halie Olson earned her bachelor’s degree in neurobiology from Harvard College in 2017. She is currently a graduate student in MIT’s Department of Brain and Cognitive Sciences working with John Gabrieli and Rebecca Saxe. She studies how early life experiences and environments impact brain development, particularly in the context of reading and language, and what this means for children’s educational outcomes.
_____
Do you have a question for The Brain? Ask it here.