Researchers at MIT’s McGovern Institute have discovered a bacterial enzyme that they say could expand scientists’ CRISPR toolkit, making it easy to cut and edit RNA with the kind of precision that, until now, has only been available for DNA editing. The enzyme, called Cas7-11, modifies RNA targets without harming cells, suggesting that in addition to being a valuable research tool, it provides a fertile platform for therapeutic applications.
“This new enzyme is like the Cas9 of RNA,” says McGovern Fellow Omar Abudayyeh, referring to the DNA-cutting CRISPR enzyme that has revolutionized modern biology by making DNA editing fast, inexpensive, and exact. “It creates two precise cuts and doesn’t destroy the cell in the process like other enzymes,” he adds.
Up until now, only one other family of RNA-targeting enzymes, Cas13, has extensively been developed for RNA targeting applications. However, when Cas13 recognizes its target, it shreds any RNAs in the cell, destroying the cell along the way. Like Cas9, Cas7-11 is part of a programmable system; it can be directed at specific RNA targets using a CRISPR guide. Abudayyeh, McGovern fellow Jonathan Gootenberg, and their colleagues discovered Cas7-11 through a deep exploration of the CRISPR systems found in the microbial world. Their findings are reported today in the journal Nature.
Exploring natural diversity
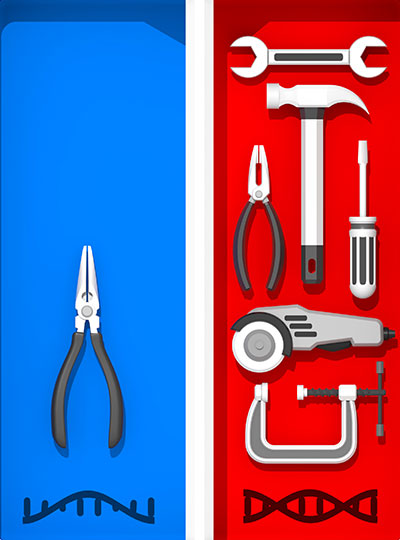
Like other CRISPR proteins, Cas7-11 is used by bacteria as a defense mechanism against viruses. After encountering a new virus, bacteria that employ the CRISPR system keep a record of the infection in the form of a small snippet of the pathogen’s genetic material. Should that virus reappear, the CRISPR system is activated, guided by a small piece of RNA to destroy the viral genome and eliminate the infection.
These ancient immune systems are widespread and diverse, with different bacteria deploying different proteins to counter their viral invaders.
“Some target DNA, some target RNA. Some are very efficient in cleaving the target but have some toxicity, and others do not. They introduce different types of cuts, they can differ in specificity—and so on,” says Eugene Koonin, an evolutionary biologist at the National Center for Biotechnology Information.
Abudayyeh, Gootenberg, and Koonin have been scouring genome sequences to learn about the natural diversity of CRISPR systems—and to mine them for potential tools. The idea, Abudayyeh says, is to take advantage of the work that evolution has already done in engineering protein machines.
“We don’t know what we’ll find,” Abudayyeh says, “but let’s just explore and see what’s out there.”
As the team was poring through public databases to examine the components of different bacterial defense systems, a protein from a bacterium that had been isolated from Tokyo Bay caught their attention. Its amino acid sequence indicated that it belonged to a class of CRISPR systems that use large, multiprotein machines to find and cleave their targets. But this protein appeared to have everything it needed to carry out the job on its own. Other known single-protein Cas enzymes, including the Cas9 protein that has been widely adopted for DNA editing, belong to a separate class of CRISPR systems—but Cas7-11 blurs the boundaries of the CRISPR classification system, Koonin says.
The enzyme, which the team eventually named Cas7-11, was attractive from an engineering perspective, because single proteins are easier to deliver to cells and make better tools than their complex counterparts. But its composition also signaled an unexpected evolutionary history. The team found evidence that through evolution, the components of a more complex Cas machine had fused together to make the Cas7-11 protein. Gootenberg equates this to discovering a bat when you had previously assumed that birds are the only animals that fly, thereby recognizing that there are multiple evolutionary paths to flight. “It totally changes the landscape of how these systems are thought about, both functionally and evolutionarily,” he says.
Precision editing
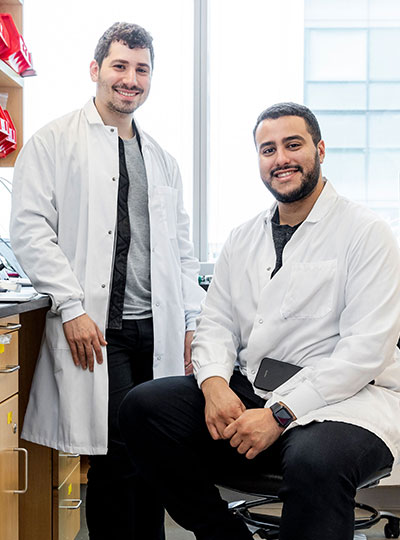
When Gootenberg and Abudayyeh produced the Cas7-11 protein in their lab and began experimenting with it, they realized this unusual enzyme offered a powerful means to manipulate and study RNA. When they introduced it into cells along with an RNA guide, it made remarkably precise cuts, snipping its targets while leaving other RNA undisturbed. This meant they could use Cas7-11 to change specific letters in the RNA code, correcting errors introduced by genetic mutations. They were also able to program Cas7-11 to either stabilize or destroy particular RNA molecules inside cells, which gave them the ability to adjust the levels of the proteins encoded by those RNAs.
Abudayyeh and Gootenberg also found that Cas7-11’s ability to cut RNA could be dampened by a protein that appeared likely to also be involved in triggering programmed cell death, suggesting a possible link between CRISPR defense and a more extreme response to infection.
The team showed that a gene therapy vector can deliver the complete Cas7-11 editing system to cells and that Cas7-11 does not compromise cells’ health. They hope that with further development, the enzyme might one day be used to edit disease-causing sequences out of a patient’s RNA so their cells can produce healthy proteins, or to dial down the level of a protein that is doing harm due to genetic disease.
“We think that the unique way that Cas7-11 cuts enables many interesting and diverse applications,” Gootenberg says, noting that no other CRISPR tool cuts RNA so precisely. “It’s yet another great example of how these basic-biology driven explorations can yield new tools for therapeutics and diagnostics,” he adds. “And we’re certainly still just scratching the surface of what’s out there in natural diversity.”