Michal De-Medonsa, technical associate and manager of the Jazayeri lab, created a large wood mosaic for her lab. We asked Michal to tell us a bit about the mosaic, her inspiration, and how in the world she found the time to create such an exquisitely detailed piece of art.
______
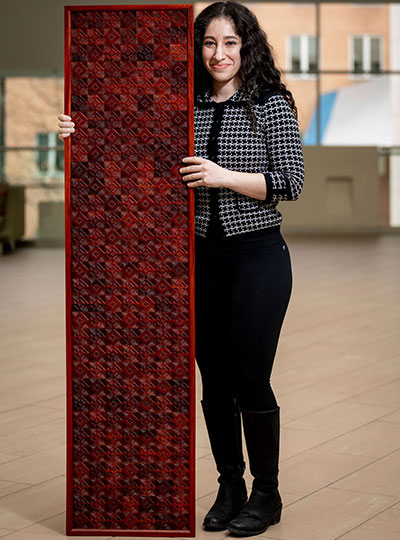
Describe this piece of art for us.
To make a piece this big (63″ x 15″), I needed several boards of padauk wood. I could have just etched each board as a whole unit and glued the 13 or so boards to each other, but I didn’t like the aesthetic. The grain and color within each board would look beautiful, but the line between each board would become obvious, segmented, and jarring when contrasted with the uniformity within each board. Instead, I cut out about 18 separate squares out of each board, shuffled all 217 pieces around, and glued them to one another in a mosaic style with a larger pattern (inspired by my grandfather’s work in granite mosaics).
What does this mosaic mean to you?
Once every piece was shuffled, the lines between single squares were certainly visible, but as a feature, were far less salient than had the full boards been glued to one another. As I was working on the piece, I was thinking about how the same concept holds true in society. Even if there is diversity within a larger piece (an institution, for example), there is a tendency for groups to form within the larger piece (like a full board), diversity becomes separated. This isn’t a criticism of any institution, it is human nature to form in-groups. It’s subconscious (so perhaps the criticism is that we, as a society, don’t give that behavior enough thought and try to ameliorate our reflex to group with those who are “like us”). The grain of the wood is uniform, oriented in the same direction, the two different cutting patterns create a larger pattern within the piece, and there are smaller patterns between and within single pieces. I love creating and finding patterns in my art (and life). Alfred North Whitehead wrote that “understanding is the apperception of pattern as such.” True, I believe, in science, art, and the humanities. What a great goal – to understand.
Tell us about the name of this piece.
Every large piece I make is inspired by the people I make it for, and is therefore named after them. This piece is called JazLab. Having lived around the world, and being a descendant of a nomadic people, I don’t consider any one place home, but am inspired by every place I’ve lived. In all of my work, you can see elements of my Jewish heritage, antiquity, the Middle East, Africa, and now MIT.
How has MIT influenced your art?
MIT has influenced me in the most obvious way MIT could influence anyone – technology. Before this series, I made very small versions of this type of work, designing everything on a piece of paper with a pencil and a ruler, and making every cut by hand. Each of those small squares would take ~2 hours (depending on the design), and I was limited to softer woods.
Since coming to MIT, I learned that I had access to the Hobby Shop with a huge array of power tools and software. I began designing my patterns on the computer and used power tools to make the cuts. I actually struggled a lot with using the tech – not because it was hard (which, it really is when you just start out), but rather because it felt like I was somehow “cheating.” How is this still art? And although this is something I still think about often, I’ve tried to look at it in this way: every generation, in their time, used the most advanced technology. The beauty and value of the piece doesn’t come from how many bruises, cuts, and blisters your machinery gave you, or whether you scraped the wood out with your nails, but rather, once you were given a tool, what did you decide to do with it? My pieces still have a huge hand-on-material work, but I am working on accepting that using technology in no way devalues the work.
Given your busy schedule with the Jazayeri lab, how did you find the time to create this piece of art?
I took advantage of any free hour I could. Two days out of the week, the hobby shop is open until 9pm, and I would additionally go every Saturday. For the parts that didn’t require the shop (adjusting each piece individually with a carving knife, assembling them, even most of the glueing) I would just work at home – often very late into the night.
______
JazLab is on display in the Jazayeri lab in MIT Bldg 46.