This story also appears in the Spring 2024 issue of BrainScan.
___
Like many people, graduate student Guillermo Herrera-Arcos found himself working from home in the spring of 2020. Surrounded by equipment he’d hastily borrowed from the lab, he began testing electrical components he would need to control muscles in a new way. If it worked, he and colleagues in Hugh Herr’s lab might have found a promising strategy for restoring movement when signals from the brain fail to reach the muscles, such as after a spinal cord injury or stroke.

Herrera-Arcos and Herr’s work is one way McGovern neuroscientists are working at the interface of brain and machine. Such work aims to enable better ways of understanding and treating injury and disease, offering scientists tools to manipulate neural signaling as well as to replace its function when it is lost.
Restoring movement
The system Herrera-Arcos and Herr were developing wouldn’t be the first to bypass the brain to move muscles. Neuroprosthetic devices that use electricity to stimulate muscle-activating motor neurons are sometimes used during rehabilitation from an injury, helping patients maintain muscle mass when they can’t use their muscles on their own. But existing neuroprostheses lack the precision of the body’s natural movement system. They send all-or-nothing signals that quickly tire muscles out.
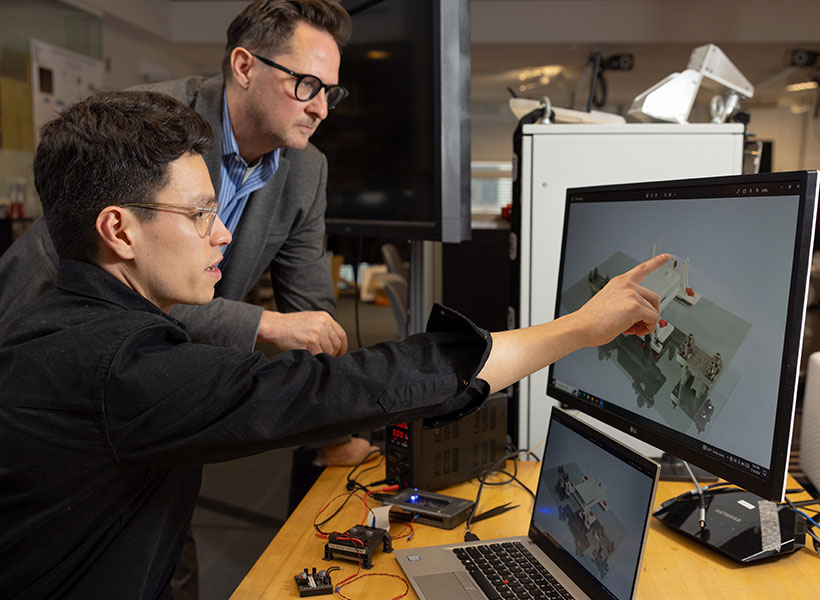
Researchers attribute that fatigue to an unnatural recruitment of neurons and muscle fibers. Electrical signals go straight to the largest, most powerful components of the system, even when smaller units could do the job. “You turn up the stimulus and you get no force, and then suddenly, you get too much force. And then fatigue, a lack of controllability, and so on,” Herr explains. The nervous system, in contrast, calls first on small motor units and recruits larger ones only when needed to generate more force.
Optical solution
In hopes of recreating this strategic pattern of muscle activation, Herr and Herrera-Arcos turned to a technique pioneered by McGovern Investigator Edward Boyden that has become common research: controlling neural activity with light. To put neurons under their control, researchers equip them with light-sensitive proteins. The cells can then be switched on or off within milliseconds using an optic fiber.
When a return to the lab enabled Herr and Herrera-Arcos to test their idea, they were thrilled with the results. Using light to switch on motor neurons and stimulate a single muscle in mice, they recreated the nervous system’s natural muscle activation pattern. Consequently, fatigue did not set in nearly as quickly as it would with an electrically-activated system. Herrera-Arcos says he set out to measure the force generated by the muscle and how long it took to fatigue, and he had to keep extending his experiments: After an hour of light stimulation, it was still going strong.
To optimize the force generated by the system, the researchers used feedback from the muscle to modulate the intensity of the neuron-activating light. Their success suggests this type of closed-loop system could enable fatigue-resistant neuroprostheses for muscle control.
“The field has been struggling for many decades with the challenge of how to control living muscle tissue,” Herr says. “So the idea that this could be solved is very, very exciting.”
There’s work to be done to translate what the team has learned into practical neuroprosthetics for people who need them. To use light to stimulate human motor neurons, light-sensitive proteins will need to be delivered to those cells. Figuring out how to do that safely is a high priority at the K. Lisa Yang Center for Bionics, which Herr co-directs with Boyden, and might lead to better ways of obtaining tactile and proprioceptive feedback from prosthetic limbs, as well as to control muscles for the restoration of natural movements after spinal cord injury. “It would be a game changer for a number of conditions,” Herr says.
Gut-brain connection
While Herr’s team works where the nervous system meets the muscle, researchers in Polina Anikeeva’s lab are exploring the brain’s relationship with an often-overlooked part of the nervous system — the hundreds of millions of neurons in the gut.
“Classically, when we think of brain function in neuroscience, it is always studied in the framework of how the brain interacts with the surrounding environment and how it integrates different stimuli,” says Atharva Sahasrabudhe, a graduate student in the group. “But the brain does not function in a vacuum. It’s constantly getting and integrating signals from the peripheral organs.”
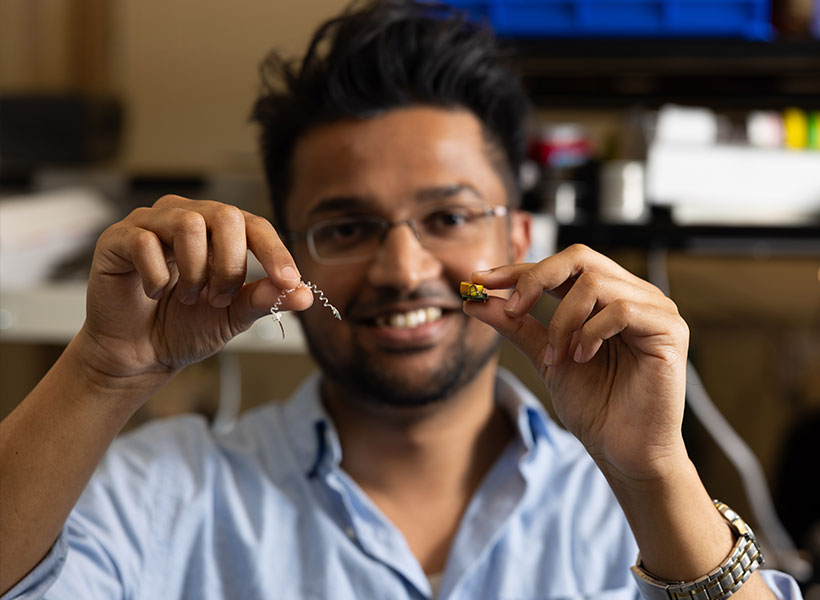
The nervous system has a particularly pronounced presence in the gut. Neurons embedded within the walls of the gastrointestinal (GI) tract monitor local conditions and relay information to the brain. This mind-body connection may help explain the GI symptoms associated with some brain-related conditions, including Parkinson’s disease, mood disorders, and autism. Researchers have yet to untangle whether GI symptoms help drive these conditions, are a consequence of them, or are coincidental. Either way, Anikeeva says, “if there is a GI connection, maybe we can tap into this connection to improve the quality of life of affected individuals.”
Flexible fibers
At the K. Lisa Yang Brain-Body Center that Anikeeva directs, studying how the gut communicates with the brain is a high priority. But most of neuroscientists’ tools are designed specifically to investigate the brain. To explore new territory, Sahasrabudhe devised a device that is compatible with the long and twisty GI tract of a mouse.
The new tool is a slender, flexible fiber equipped with light emitters for activating subsets of cells and tiny channels for delivering nutrients or drugs. To access neurons dispersed throughout the GI tract, its wirelessly controlled components are embedded along its length. A more rigid probe at one end of the device is designed to monitor and manipulate neural activity in the brain, so researchers can follow the nervous system’s swift communications across the gut-brain axis.
Scientists on Anikeeva’s team are deploying the device to investigate how gut-brain communications contribute to several conditions. Postdoctoral researcher Sharmelee Selvaraji is focused on Parkinson’s disease. Like many scientists, she wonders whether the neurodegenerative movement disorder might actually start in the gut. There’s a molecular link: the misshapen protein that sickens brain cells in patients with Parkinson’s disease has been found aggregating in the gut, too. And the constipation and other GI problems that are common complaints for people with Parkinson’s disease usually start decades before the onset of motor symptoms. She hopes that by investigating gut-brain communications in a mouse model of the disease, she will uncover important clues about its origins and progression.
“We’re trying to observe the effects of Parkinson’s in the gut, and then eventually, we may be able to intervene at an earlier stage to slow down the disease progression, or even cure it,” says Selvaraji.
Meanwhile, colleagues in the lab are exploring related questions about gut-brain communications in mouse models of autism, anxiety disorders, and addiction. Others continue to focus on technology development, adding new capabilities to the gut-brain probe or applying similar engineering principles to new problems.
“We are realizing that the brain is very much connected to the rest of the body,” Anikeeva says. “There is now a lot of effort in the lab to create technology suitable for a variety of really interesting organs that will help us study brain-body connections.”