Making and breaking habits is a prime function of the striatum, a large forebrain region that underlies the cerebral cortex. McGovern researchers have identified a particular gene that controls striatal function as well as repetitive behaviors that are linked to drug addiction vulnerability.
To identify genes involved specifically in striatal functions, MIT Institute Professor Ann Graybiel previously identified genes that are preferentially expressed in striatal neurons. One identified gene encodes CalDAG-GEFI (CDGI), a signaling molecule that effects changes inside of cells in response to extracellular signals that are received by receptors on the cell surface. In a paper to be published in the October issue of Neurobiology of Disease and now available online, Graybiel, along with former Research Scientist Jill Crittenden and collaborators James Surmeier and Shenyu Zhai at the Feinman School of Medicine at Northwestern University, show that CDGI is key for controlling behavioral responses to drugs of abuse and underlying neuronal plasticity (cellular changes induced by experience) in the striatum.
“This paper represents years of intensive research, which paid off in the end by identifying a specific cellular signaling cascade for controlling repetitive behaviors and neuronal plasticity,” says Graybiel, who is also an investigator at the McGovern Institute and a professor of brain and cognitive sciences at MIT.
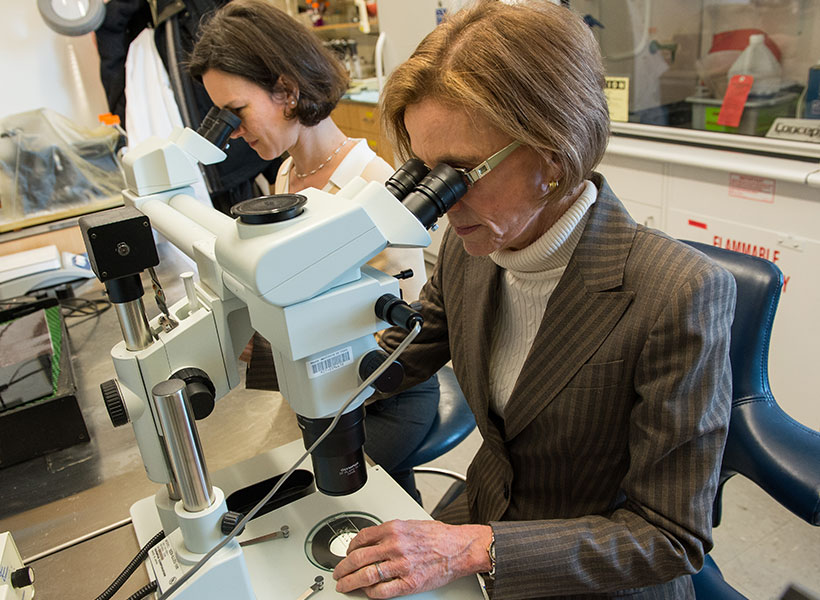
Surprise discovery
To understand the essential roles of CDGI, Crittenden first engineered “knockout” mice that lack the gene encoding CDGI. Then the Graybiel team began looking for abnormalities in the CDGI knockout mice that could be tied to the loss of CDGI’s function.
Initially, they noticed that the rodent ear-tag IDs often fell off in the knockout mice, an observation that ultimately led to the surprise discovery by the Graybiel team and others that CDGI is expressed in blood platelets and is responsible for a bleeding disorder in humans, dogs, and other animals. The CDGI knockout mice were otherwise healthy and seemed just like their “wildtype” brothers and sisters, which did not carry the gene mutation. To figure out the role of CDGI in the brain, the Graybiel team would have to scrutinize the mice more closely.
Challenging the striatum
Both the CDGI knockout and wildtype mice were given an extensive set of behavioral and neurological tests and the CDGI mice showed deficits in two tests designed to challenge the striatum.
In one test, mice must find their way through a maze by relying on egocentric (i.e. self-referential) cues, such as their turning right or turning left, and not competing allocentric (i.e. external) cues, such as going toward a bright poster on the wall. Egocentric cues are thought to be processed by the striatum whereas allocentric cues are thought to rely on the hippocampus.
In a second test of striatal function, mice learned various gait patterns to match different patterns of rungs on their running wheel, a task designed to test the mouse’s ability to learn and remember a motor sequence.
The CDGI mice learned both of these striatal tasks more slowly than their wildtype siblings, suggesting that the CDGI mice might perform normally in general tests of behavior because they are able to compensate for striatal deficits by using other brain regions such as the hippocampus to solve standard tasks.
The team then decided to give the mice a completely different type of test that relies on the striatum. Because the striatum is strongly activated by drugs of abuse, which elevate dopamine and drive motor habits, Crittenden and collaborator Morgane Thomsen (now at the University of Copenhagen) looked to see whether the CDGI knockout mice respond normally to amphetamine and cocaine.
Psychomotor stimulants like cocaine and amphetamine normally induce a mixture of hyperactive behaviors such as pacing and focused repetitive behaviors like skin-picking (also called stereotypy or punding in humans). The researchers found however, that the drug-induced behaviors in the CDGI knockout mice were less varied than the normal mice and consisted of abnormally prolonged stereotypy, as though the mice were unable to switch between behaviors. The researchers were able to map the abnormal behavior to CDGI function in the striatum by showing that the same vulnerability to drug-induced stereotypy was observed in mice that were engineered to delete CDGI in the striatum after birth (“conditional knockouts”), but to otherwise have normal CDGI throughout the body.
Controlling cravings
In addition to exhibiting prolonged, repetitive behaviors, the CDGI knockout mice had a vulnerability to self-administer drugs. Although previous research had shown that treatments that activate the M1 acetylcholine receptor can block cocaine self-administration, the team found that this therapy was ineffective in CDGI knockout mice. Knockouts continued to self-administer cocaine (suggesting increased craving for the drug) at the same rate before and after M1 receptor activation treatment, even though the treatment succeeded with their sibling control mice. The researchers concluded that CDGI is critically important for controlling repetitive behaviors and the ability to stop self-administration of addictive stimulants.
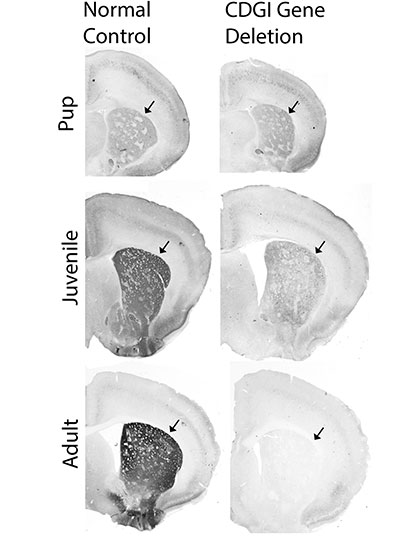
To better understand how CDGI is linked to the M1 receptor at the cellular level, the team turned to slice physiologists, scientists who record the electrical activity of neurons in brain slices. Their recordings showed that striatal neurons from CDGI knockouts fail to undergo the normal, expected electrophysiological changes after receiving treatments that target the M1 receptor. In particular, the neurons of the striatum that function broadly to stop ongoing behaviors, did not integrate cellular signals properly and failed to undergo “long-term potentiation,” a type of neuronal plasticity thought to underlie learning.
The new findings suggest that excessive repetitive movements are controlled by M1 receptor signaling through CDGI in indirect pathway neurons of the striatum, a neuronal subtype that degenerates in Huntington’s disease and is affected by dopamine loss and l-DOPA replacement therapy in Parkinson’s disease.
“The M1 acetylcholine receptor is a target for therapeutic drug development in treating cognitive and behavioral problems in multiple disorders, but progress has been severely hampered by off-target side-effects related to the wide-spread expression of the M1 receptor,” Graybiel explains. “Our findings suggest that CDGI offers the possibility for forebrain-specific targeting of M1 receptor signaling cascades that are of interest for blocking pathologically repetitive and unwanted behaviors that are common to numerous brain disorders including Huntington’s disease, drug addiction, autism, and schizophrenia as well as drug-induced dyskinesias. We hope that this work can help therapeutic development for these major health problems.”
This work was funded by the James W. (1963) and Patricia T. Poitras Fund, the William N. & Bernice E. Bumpus Foundation, the Saks Kavanaugh Foundation, the Simons Foundation, and the National Institute of Health.