A deepening understanding of the brain has created unprecedented opportunities to alleviate the challenges posed by disability. Scientists and engineers are taking design cues from biology itself to create revolutionary technologies that restore the function of bodies affected by injury, aging, or disease – from prosthetic limbs that effortlessly navigate tricky terrain to digital nervous systems that move the body after a spinal cord injury.
With the establishment of the new K. Lisa Yang Center for Bionics, MIT is pushing forward the development and deployment of enabling technologies that communicate directly with the nervous system to mitigate a broad range of disabilities. The center’s scientists, clinicians, and engineers will work together to create, test, and disseminate bionic technologies that integrate with both the body and mind.
The center is funded by a $24 million gift to MIT’s McGovern Institute for Brain Research from philanthropist Lisa Yang, a former investment banker committed to advocacy for individuals with visible and invisible disabilities.

Her previous gifts to MIT have also enabled the establishment of the K. Lisa Yang and Hock E. Tan Center for Molecular Therapeutics in Neuroscience, Hock E. Tan and K. Lisa Yang Center for Autism Research, Y. Eva Tan Professorship in Neurotechnology, and the endowed K. Lisa Yang Post-Baccalaureate Program.
“The K. Lisa Yang Center for Bionics will provide a dynamic hub for scientists, engineers and designers across MIT to work together on revolutionary answers to the challenges of disability,” says MIT President L. Rafael Reif. “With this visionary gift, Lisa Yang is unleashing a powerful collaborative strategy that will have broad impact across a large spectrum of human conditions – and she is sending a bright signal to the world that the lives of individuals who experience disability matter deeply.”
An interdisciplinary approach
To develop prosthetic limbs that move as the brain commands or optical devices that bypass an injured spinal cord to stimulate muscles, bionic developers must integrate knowledge from a diverse array of fields—from robotics and artificial intelligence to surgery, biomechanics, and design. The K. Lisa Yang Center for Bionics will be deeply interdisciplinary, uniting experts from three MIT schools: Science, Engineering, and Architecture and Planning. With clinical and surgical collaborators at Harvard Medical School, the center will ensure that research advances are tested rapidly and reach people in need, including those in traditionally underserved communities.
To support ongoing efforts to move toward a future without disability, the center will also provide four endowed fellowships for MIT graduate students working in bionics or other research areas focused on improving the lives of individuals who experience disability.
“I am thrilled to support MIT on this major research effort to enable powerful new solutions that improve the quality of life for individuals who experience disability,” says Yang. “This new commitment extends my philanthropic investment into the realm of physical disabilities, and I look forward to the center’s positive impact on countless lives, here in the US and abroad.”
The center will be led by Hugh Herr, a professor of media arts and sciences at MIT’s Media Lab, and Ed Boyden, the Y. Eva Tan Professor of Neurotechnology at MIT, a professor of biological engineering, brain and cognitive sciences, and media arts and sciences, and an investigator at MIT’s McGovern Institute and the Howard Hughes Medical Institute.
A double amputee himself, Herr is a pioneer in the development of bionic limbs to improve mobility for those with physical disabilities. “The world profoundly needs relief from the disabilities imposed by today’s nonexistent or broken technologies. We must continually strive towards a technological future in which disability is no longer a common life experience,” says Herr. “I am thrilled that the Yang Center for Bionics will help to measurably improve the human experience for so many.”
Boyden, who is a renowned creator of tools to analyze and control the brain, will play a key role in merging bionics technologies with the nervous system. “The Yang Center for Bionics will be a research center unlike any other in the world,” he says. “A deep understanding of complex biological systems, coupled with rapid advances in human-machine bionic interfaces, mean we will soon have the capability to offer entirely new strategies for individuals who experience disability. It is an honor to be part of the center’s founding team.”
Center priorities
In its first four years, the K. Lisa Yang Center for Bionics will focus on developing and testing three bionic technologies:
- Digital nervous system: to eliminate movement disorders caused by spinal cord injuries, using computer-controlled muscle activations to control limb movements while simultaneously stimulating spinal cord repair
- Brain-controlled limb exoskeletons: to assist weak muscles and enable natural movement for people affected by stroke or musculoskeletal disorders
- Bionic limb reconstruction: to restore natural, brain-controlled movements as well as the sensation of touch and proprioception (awareness of position and movement) from bionic limbs
A fourth priority will be developing a mobile delivery system to ensure patients in medically underserved communities have access to prosthetic limb services. Investigators will field test a system that uses a mobile clinic to conduct the medical imaging needed to design personalized, comfortable prosthetic limbs and to fit the prostheses to patients where they live. Investigators plan to initially bring this mobile delivery system to Sierra Leone, where thousands of people suffered amputations during the country’s 11-year civil war. While the population of persons with amputation continues to increase each year in Sierra Leone, today less than 10% of persons in need benefit from functional prostheses. Through the mobile delivery system, a key center objective is to scale up production and access of functional limb prostheses for Sierra Leoneans in dire need.
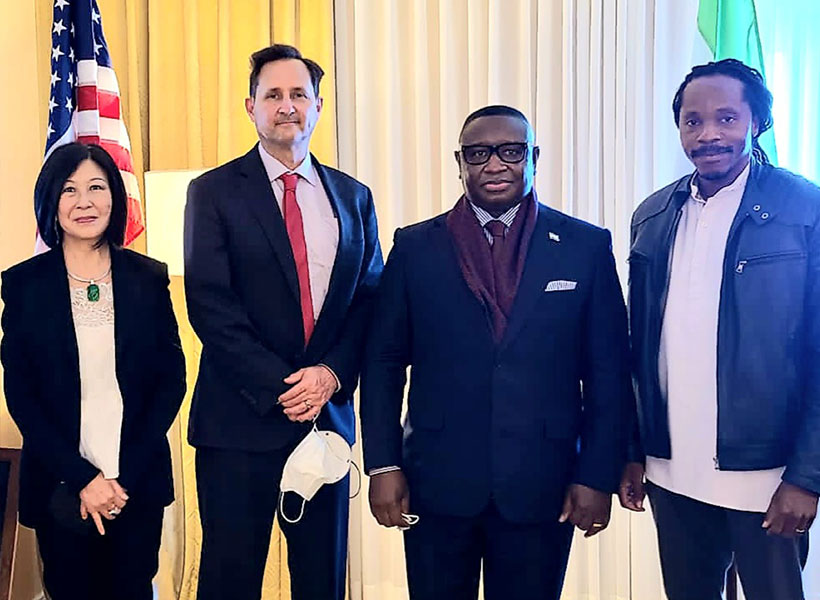
“The mobile prosthetics service fueled by the K. Lisa Yang Center for Bionics at MIT is an innovative solution to a global problem,” said Julius Maada Bio, President of Sierra Leone. “I am proud that Sierra Leone will be the first site for deploying this state-of-the-art digital design and fabrication process. As leader of a government that promotes innovative technologies and prioritizes human capital development, I am overjoyed that this pilot project will give Sierra Leoneans (especially in rural areas) access to quality limb prostheses and thus improve their quality of life.”
Together, Herr and Boyden will launch research at the bionics center with three other MIT faculty: Assistant Professor of Media Arts and Sciences Canan Dagdeviren, Walter A. Rosenblith Professor of Cognitive Neuroscience Nancy Kanwisher, and David H. Koch (1962) Institute Professor Robert Langer. They will work closely with three clinical collaborators at Harvard Medical School: orthopedic surgeon Marco Ferrone, plastic surgeon Matthew Carty, and Nancy Oriol, Faculty Associate Dean for Community Engagement in Medical Education.
“Lisa Yang and I share a vision for a future in which each and every person in the world has the right to live without a debilitating disability if they so choose,” adds Herr. “The Yang Center will be a potent catalyst for true innovation and impact in the bionics space, and I am overjoyed to work with my colleagues at MIT, and our accomplished clinical partners at Harvard, to make important steps forward to help realize this vision.”